2.1 Atoms, Isotopes, Ions, and Molecules: The Building Blocks
Learning Objectives
By the end of this section, you will be able to:
- Describe matter and elements
- Describe the interrelationship between protons, neutrons, and electrons, and the ways in which electrons can be donated or shared between atoms
The Chemical Foundation of Life
Watch a video about electrons and how the electrons in chemical bonds influence the shape and function of molecules.
At its most fundamental level, life is made up of matter. Matter occupies space and has mass. All matter is composed of elements, substances that cannot be broken down or transformed chemically into other substances. Each element is made of atoms, each with a constant number of protons and unique properties. A total of 118 elements have been defined; however, only 98 occur naturally, and fewer than 30 are found in living cells. The remaining 26 elements are unstable and, therefore, do not exist for very long or are theoretical and have yet to be detected. These unique properties allow elements to combine and to bond with each other in specific ways.
Each element is designated by its chemical symbol, which is a single capital letter or, when the first letter is already “taken” by another element, a combination of two letters. Some elements follow the English term for the element, such as C for carbon and Ca for calcium. Other elements’ chemical symbols derive from their Latin names. For example, the symbol for sodium is Na, referring to natrium, the Latin word for sodium.
The four elements common to all living organisms are oxygen (O), carbon (C), hydrogen (H), and nitrogen (N). In the nonliving world, elements are found in different proportions, and some elements common to living organisms are relatively rare on the earth as a whole, as Table 2.1 shows. For example, the atmosphere is rich in nitrogen and oxygen but contains little carbon and hydrogen, while the earth’s crust, although it contains oxygen and a small amount of hydrogen, has little nitrogen and carbon. In spite of their differences in abundance, all elements and the chemical reactions between them obey the same chemical and physical laws regardless of whether they are a part of the living or nonliving world.
Table 2.1 Approximate Percentage of Elements in Living Organisms (Humans) Compared to the Nonliving World
Element | Life (Humans) | Atmosphere | Earth’s Crust |
Oxygen (O) | 65% | 21% | 46% |
Carbon (C) | 18% | trace | trace |
Hydrogen (H) | 10% | trace | 0.1% |
Nitrogen (N) | 3% | 78% | trace |
Atoms
An atom is the smallest component of an element that retains all of the chemical properties of that element. For example, one hydrogen atom has all of the properties of the element hydrogen, such as it exists as a gas at room temperature, and it bonds with oxygen to create a water molecule. Hydrogen atoms cannot be broken down into anything smaller while still retaining the properties of hydrogen. If a hydrogen atom were broken down into subatomic particles, it would no longer have the properties of hydrogen. At the most basic level, all organisms are made of a combination of elements. They contain atoms that combine together to form molecules. In multicellular organisms, such as animals, molecules can interact to form cells that combine to form tissues, which make up organs. These combinations continue until entire multicellular organisms are formed.
All atoms contain protons, neutrons, and electrons. The only exception is the most common isotope of hydrogen (H), whose atom is made of one proton and one electron. (Isotopes are discussed later on.) Protons and neutrons reside in the nucleus (the core) of an atom. Electrons travel in the space around the nucleus. The locations of protons, neutrons, and electrons in an atom are shown in Figure 2.1.
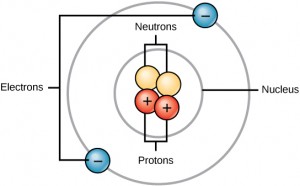
Protons and neutrons have approximately the same mass, about 1.67 × 10-24 grams. Scientists arbitrarily define this amount of mass as 1 atomic mass unit (amu) or one dalton (Da), as Table 2.2 shows. Although similar in mass, protons and neutrons differ in their electric charge. A proton is positively charged; whereas, a neutron is uncharged. Therefore, the number of neutrons in an atom contributes significantly to its mass, but not to its charge. Electrons are much smaller in mass than protons, weighing only 9.11 × 10-28 grams, or about 1/1800 amu. Hence, electrons do not contribute much to an element’s overall atomic mass. Therefore, when considering atomic mass, it is customary to ignore the mass of any electrons and calculate the atom’s mass based on the number of protons and neutrons alone. Although not significant contributors to mass, electrons do contribute greatly to the atom’s charge, as each electron has a negative charge equal in magnitude to the proton’s positive charge. In uncharged, neutral atoms, the number of electrons orbiting the nucleus is equal to the number of protons inside the nucleus. In these atoms, the positive and negative charges cancel each other out, leading to an atom with no net charge.
Charge | Mass (amu) | Location | |
Proton | +1 | 1 | nucleus |
Neutron | 0 | 1 | nucleus |
Electron | -1 | 0 | orbitals |
Accounting for the sizes of protons, neutrons, and electrons, most of the atom’s volume—greater than 99 percent—is empty space. With all this empty space, one might ask why so-called solid objects do not just pass through one another. The reason they do not is that the electrons that surround all atoms are negatively charged and like charges repel each other.
Atomic Number and Mass
Atoms of each element contain a characteristic number of protons and electrons. The number of protons determines an element’s atomic number, which scientists use to distinguish one element from another. The number of neutrons is variable, resulting in isotopes, which are different forms of the same atom that vary only in the number of neutrons they possess. Together, the number of protons and neutrons determine an element’s mass number, as Figure 2.2 illustrates. Note that we disregard the small contribution of mass from electrons in calculating the mass number. We can use this approximation of mass to easily calculate how many neutrons an element has by simply subtracting the number of protons from the mass number. Since an element’s isotopes will have slightly different mass numbers, scientists also determine the atomic mass, which is the calculated mean of the mass number for its naturally occurring isotopes. Often, the resulting number contains a fraction. For example, the atomic mass of chlorine (Cl) is 35.45 because chlorine is composed of several isotopes, some (the majority) with atomic mass 35 (17 protons and 18 neutrons) and some with atomic mass 37 (17 protons and 20 neutrons).
VISUAL CONNECTION
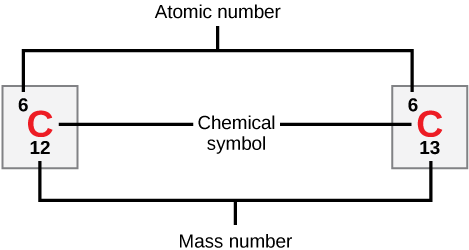
How many neutrons do carbon-12 and carbon-13 have, respectively?
These numbers provide information about the elements and how they will react when combined. Different elements have different melting and boiling points, and are in different states (liquid, solid, or gas) at room temperature. They also combine in different ways. Some form specific types of bonds, whereas others do not. How they combine is based on the number of electrons present. Because of these characteristics, the elements are arranged into the periodic table of elements (Figure 2.3), a chart of the elements that includes the atomic number and relative atomic mass of each element. The periodic table also provides key information about the properties of elements —often indicated by color-coding. The arrangement of the table also shows how the electrons in each element are organized and provides important details about how atoms will react with each other to form molecules.
Isotopes are different forms of the same element that have the same number of protons, but a different number of neutrons. Some elements, such as carbon, potassium, and uranium, have naturally occurring isotopes. Carbon-12, the most common isotope of carbon, contains six protons and six neutrons. Therefore, it has a mass number of 12 (six protons and six neutrons) and an atomic number of 6 (which makes it carbon). Carbon-14 contains six protons and eight neutrons. Therefore, it has a mass number of 14 (six protons and eight neutrons) and an atomic number of six, meaning it is still the element carbon. These two alternate forms of carbon are isotopes. Some isotopes are unstable and will lose neutrons, other subatomic particles, or energy to form more stable elements. These are called radioactive isotopes or radioisotopes.
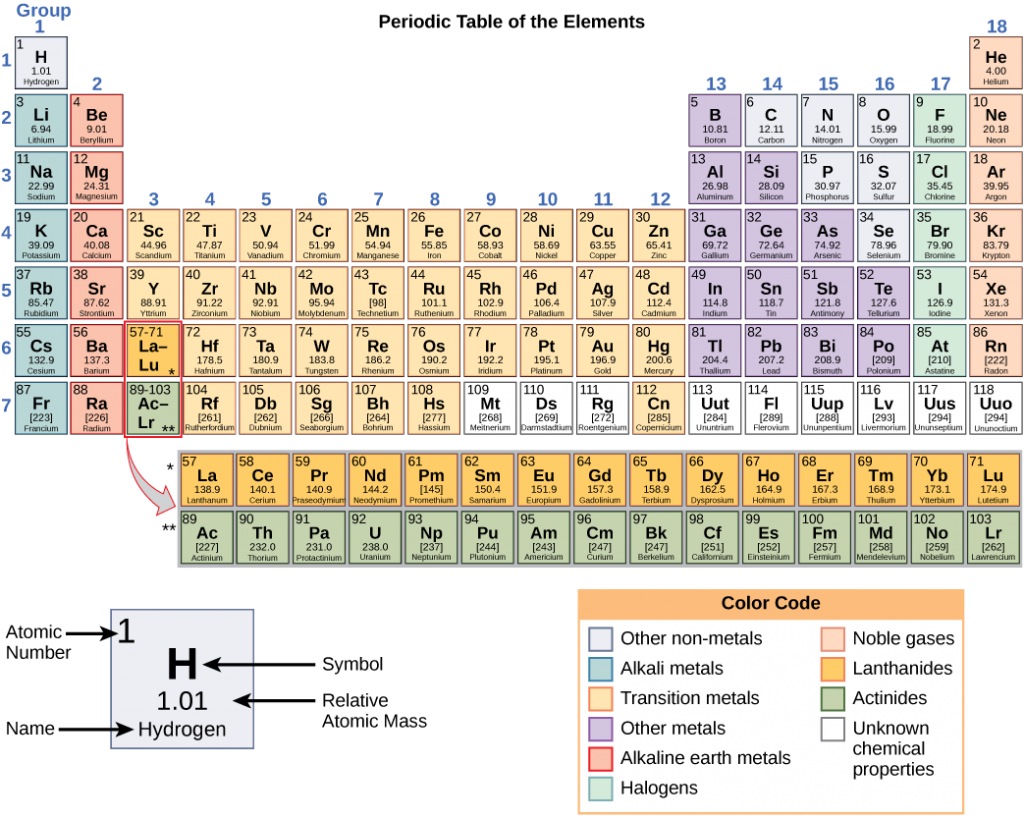
How many neutrons do (K) potassium-39 and potassium-40 have, respectively?
Electron Shells and the Bohr Model
Note that there is a connection between the number of protons in an element, the atomic number that distinguishes one element from another, and the number of electrons it has. In all electrically neutral atoms, the number of electrons is the same as the number of protons. Thus, each element, at least when electrically neutral, has a characteristic number of electrons equal to its atomic number.
In 1913, Danish scientist Niels Bohr (1885–1962) developed an early model of the atom. The Bohr model shows the atom as a central nucleus containing protons and neutrons, with the electrons in circular orbitals at specific distances from the nucleus, as Figure 2.4 illustrates. These orbits form the principal electron shells or energy levels, which are a way of visualizing the organization of electrons around the nucleus. These energy levels are designated by a number and the symbol “n.” For example, 1n represents the first energy level located closest to the nucleus.
Looking at the periodic table again, you will notice that there are seven rows. These rows correspond to the number of shells that the elements within that row have. The elements within a particular row have increasing numbers of electrons as the columns proceed from left to right. Although each element has the same number of shells, not all of the shells are completely filled with electrons. If you look at the second row of the periodic table, you will find lithium (Li), beryllium (Be), boron (B), carbon (C), nitrogen (N), oxygen (O), fluorine (F), and neon (Ne). These all have electrons that occupy only the first and second shells. Lithium has only one electron in its outermost shell, beryllium has two electrons, boron has three, and so on, until the entire shell is filled with eight electrons, as is the case with neon. Electrons fill orbitals in a consistent order: they first fill the orbitals closest to the nucleus, then they continue to fill orbitals of increasing energy further from the nucleus. If there are multiple orbitals of equal energy, they fill with one electron in each energy level before adding a second electron. The electrons of the outermost energy level, the valence shell, determine the atom’s energetic stability and its tendency to react with other atoms to form compounds.
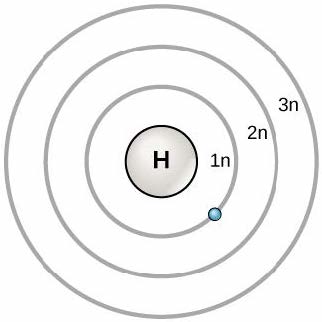
At the lowest energy configuration, ground state, atoms fill the inner shells first, often resulting in a variable number of electrons in the outermost shell. The innermost shell has a maximum of two electrons but the next two rows, the electron shells each have a maximum of eight electrons. This is known as the octet rule, which states, with the exception of the innermost shell, that atoms are more stable energetically when they have eight electrons in their valence shell, the outermost electron shell.
Figure 2.5 shows examples of some neutral atoms and their electron configurations. Hydrogen has one electron; therefore, it has only one spot occupied within the lowest shell. Helium has a complete outer electron shell, with a duet of two electrons filling its first and only shell. If you look at the periodic table, you will see that hydrogen and helium are the only two elements in the first row. This is because they only have electrons in their first shell.
The second and third energy levels can hold up to eight electrons. The eight electrons are arranged in four pairs and one position in each pair is filled with an electron before any pairs are completed. Neon has a complete outer 2n shell containing eight electrons. In contrast, chlorine and sodium have seven and one in their outer shells, respectively, but theoretically they would be more energetically stable if they followed the octet rule and had eight.
VISUAL CONNECTION
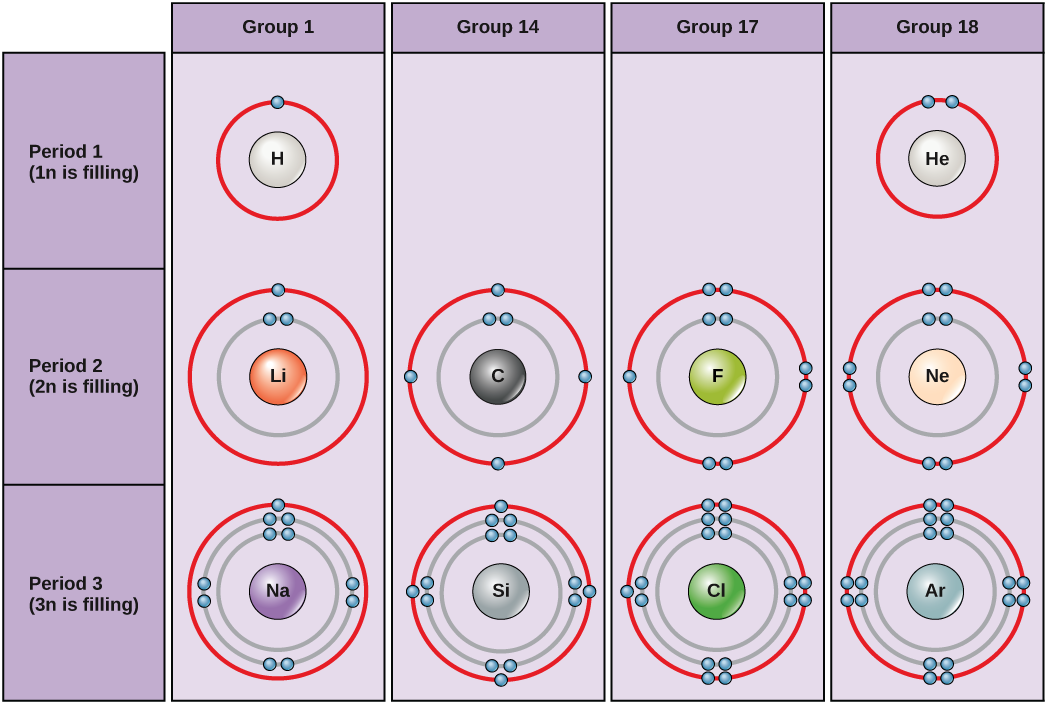
An atom may give, take, or share electrons with another atom to achieve a full valence shell, the most stable electron configuration. Looking at this figure, how many electrons do elements in Group 1 need to lose in order to achieve a stable electron configuration? How many electrons do elements in Groups 14 and 17 need to gain to achieve a stable configuration?
The columns of the periodic table relate to the number of valence electrons of the atoms at ground state. Examine more closely some of the elements in the table’s far right column in Figure 2.3. The Group 18 atoms helium (He), neon (Ne), and argon (Ar) all have filled outer electron shells, making it unnecessary for them to share electrons with other atoms to attain stability. They are highly stable as single atoms. Because they are nonreactive, scientists coin them inert (or noble gases). Compare this to the Group 1 elements in the left-hand column. These elements, including hydrogen (H), lithium (Li), and sodium (Na), all have one electron in their outermost shells. That means that they can achieve a stable configuration and a filled outer shell by donating or sharing one electron with another atom or a molecule such as water. Hydrogen will donate or share its electron to achieve this configuration, while lithium and sodium will donate their electron to become stable. As a result of losing a negatively charged electron, they become positively charged ions. Group 17 elements, including fluorine and chlorine, have seven electrons in their outermost shells, so they tend to fill this shell with an electron from other atoms or molecules, making them negatively charged ions. Group 14 elements, of which carbon is the most important to living systems, have four electrons in their outer shell allowing them to make several covalent bonds (discussed below) with other atoms. Thus, the periodic table’s columns represent the configuration of these elements’ outermost electron shells that are responsible for their similar chemical characteristics.
Electron Orbitals
Although useful to explain the reactivity and chemical bonding of certain elements, the Bohr model does not accurately reflect how electrons spatially distribute themselves around the nucleus. Electrons do not circle the nucleus like the earth orbits the sun, but we find them in electron orbitals. These relatively complex shapes result from the fact that electrons behave not just like particles, but also like waves. Mathematical equations from quantum mechanics, which scientists call wave functions, can predict within a certain level of probability where an electron might be at any given time. Scientists call the area where an electron is most likely to be found its orbital. While the concepts of electron shells, subshells, and orbitals are closely related, orbitals provide a more accurate depiction of an atom’s electron configuration because the orbital model specifies the different shapes and spatial orientations of all the places that electrons may occupy.
Recall that the Bohr model depicts an atom’s electron shell configuration. Within each electron shell are subshells, and each subshell has a specified number of orbitals each containing a maximum of two electrons. While it is impossible to calculate exactly an electron’s location, scientists know that it is most probably located within its orbital path. The letter s, p, d, and f designate the subshells. The s subshell is spherical and has one orbital. Principal shell 1n has only a single s orbital, which can hold two electrons. Principal shell 2n has one s and one p subshell, and can hold a total of eight electrons. The p subshell has three dumbbell-shaped orbitals, as Figure 2.6 illustrates. Subshells d and f have more complex shapes and contain five and seven orbitals, respectively. We do not show these in the illustration. Principal shell 3n has s, p, and d subshells and can hold 18 electrons in total. Principal shell 4n has s, p, d and f orbitals and can hold 32 electrons in total. Moving away from the nucleus, the number of electrons and orbitals in the energy levels increases. Progressing from one atom to the next in the periodic table, we can determine the electron structure by fitting an extra electron into the next available orbital for each successive element.
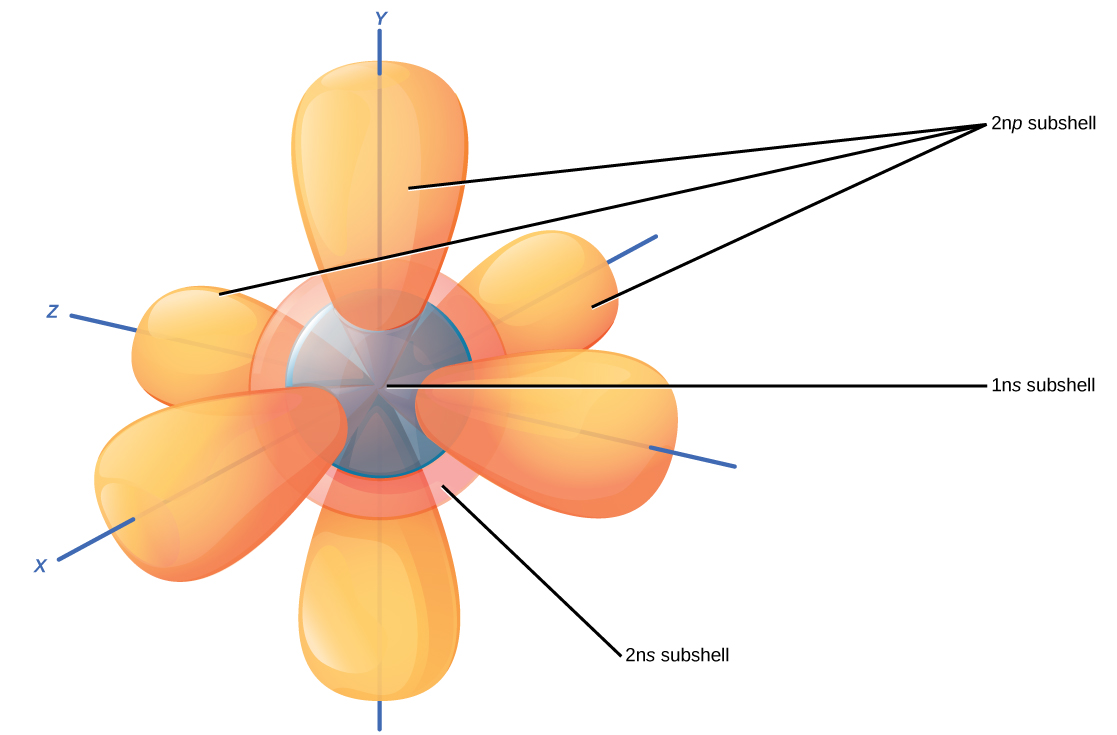
Hydrogen has one electron; therefore, it occupies only one spot within the 1s orbital. We designate this as 1s1, where the superscripted 1 refers to the one electron within the 1s orbital. Helium has two electrons; therefore, it can completely fill the 1s orbital with its two electrons. We designate this as 1s2, referring to the two electrons of helium in the 1s orbital. On the periodic table Figure 2.3, hydrogen and helium are the only two elements in the first row (period). This is because they only have electrons in their first shell, the 1s shell.
The second principal shell contains up to eight electrons in total. This shell has another spherical s orbital and three “dumbbell” shaped p orbitals, each of which can hold two electrons, as Figure 2.6 shows. After the 1s orbital fills, the second electron shell fills, first filling its 2s orbital and then its three p orbitals. When filling the p orbitals, each orbital takes a single electron. Once each p orbital has an electron, it may add a second electron. Lithium (Li) contains three electrons that occupy the first and second shells. Two electrons fill the 1s orbital, and the third electron then fills the 2s orbital. Its electron configuration is 1s22s1. Neon (Ne), alternatively, has a total of ten electrons: two are in its innermost 1s orbital and eight fill its second shell (two electrons in the 2s and six electrons among the three 2p orbitals).
LINK TO LEARNING
Watch this visual animation [Video – Atomic orbitals – electron configuration of Scadium (Z=21)] to see the spatial arrangement of the p and s orbitals.
EVOLUTION IN ACTION
Carbon Dating
Carbon-14 (14C) is a naturally occurring radioisotope that is created in the atmosphere by cosmic rays. This is a continuous process, so more 14C is always being created. As a living organism develops, the relative level of 14C in its body is equal to the concentration of 14C in the atmosphere. When an organism dies, it is no longer ingesting 14C, so the ratio will decline. 14C decays to 14N by a process called beta decay; it gives off energy in this slow process.
After approximately 5,730 years, only one-half of the starting concentration of 14C will have been converted to 14N. The time it takes for half of the original concentration of an isotope to decay to its more stable form is called its half-life. Because the half-life of 14C is long, it is used to age formerly living objects, such as fossils. Using the ratio of the 14C concentration found in an object to the amount of 14C detected in the atmosphere, the amount of the isotope that has not yet decayed can be determined. Based on this amount, the age of the fossil can be calculated to about 50,000 years. Isotopes with longer half-lives, such as potassium-40, are used to calculate the ages of older fossils. Through the use of carbon dating, scientists can reconstruct the ecology and biogeography of organisms living within the past 50,000 years (Figure 2.7).
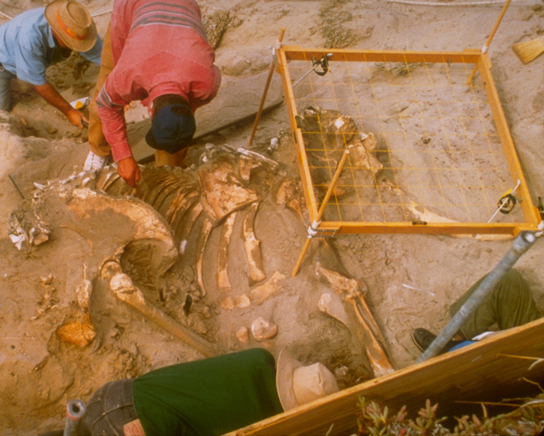
CONCEPT IN ACTION
To learn more about atoms and isotopes, and how you can tell one isotope from another, visit this site and run the simulation.
Chemical Bonding
How elements interact with one another depends on the electronic configuration of the valence shell. Elements, especially carbon, nitrogen, and oxygen, are stable when their outermost shell is filled with electrons according to the octet rule. Note that hydrogen is stabilized by a duet of electrons. This is because it is energetically favorable for atoms to be in that configuration and it makes them stable. To achieve greater stability, atoms will tend to completely fill their outer shells and will react to form bonds with the same or different elements to accomplish this goal by sharing electrons. Each chemical bond represents the sharing of two electrons. When two or more atoms chemically bond with each other, the resultant chemical structure is a molecule. The familiar water molecule, H2O, consists of two hydrogen atoms and one oxygen atom. These bond together to form water, as Figure 2.8 illustrates.
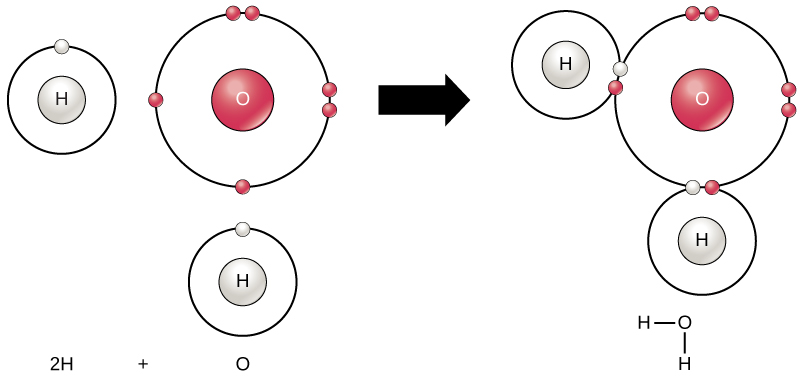
Chemical reactions occur when two or more atoms bond together to form molecules or when bonded atoms break apart. Scientists call the substances used in the beginning of a chemical reaction reactants (usually on the left side of a chemical equation), and we call the substances at the end of the reaction products (usually on the right side of a chemical equation). We typically draw an arrow between the reactants and products to indicate the chemical reaction’s direction. This direction is not always a “one-way street.” To create the water molecule above, the chemical equation would be:
2 H + O → H2O
An example of a simple chemical reaction is breaking down hydrogen peroxide (H2O2) molecules, each of which consists of two hydrogen atoms bonded to two oxygen atoms. The reactant hydrogen peroxide breaks down into water, containing one oxygen atom bound to two hydrogen atoms (H2O), and an oxygen molecule, which consists of two bonded oxygen atoms (O2). In the equation below, the reaction includes two hydrogen peroxide molecules and two water molecules. This is an example of a balanced chemical equation, wherein each element’s number of atoms is the same on each side of the equation. According to the law of conservation of matter, the number of atoms before and after a chemical reaction should be equal, such that no atoms are, under normal circumstances, created or destroyed.
2 H2O2 (hydrogen peroxide) → 2 H2O (water) + O2 (oxygen)
Even though all of the reactants and products of this reaction are molecules (each atom remains bonded to at least one other atom), in this reaction only hydrogen peroxide and water are representatives of compounds: they contain atoms of more than one type of element. Molecular oxygen, alternatively, as Figure 2.9 shows, consists of two double bonded oxygen atoms and is not classified as a compound but as a homonuclear molecule.
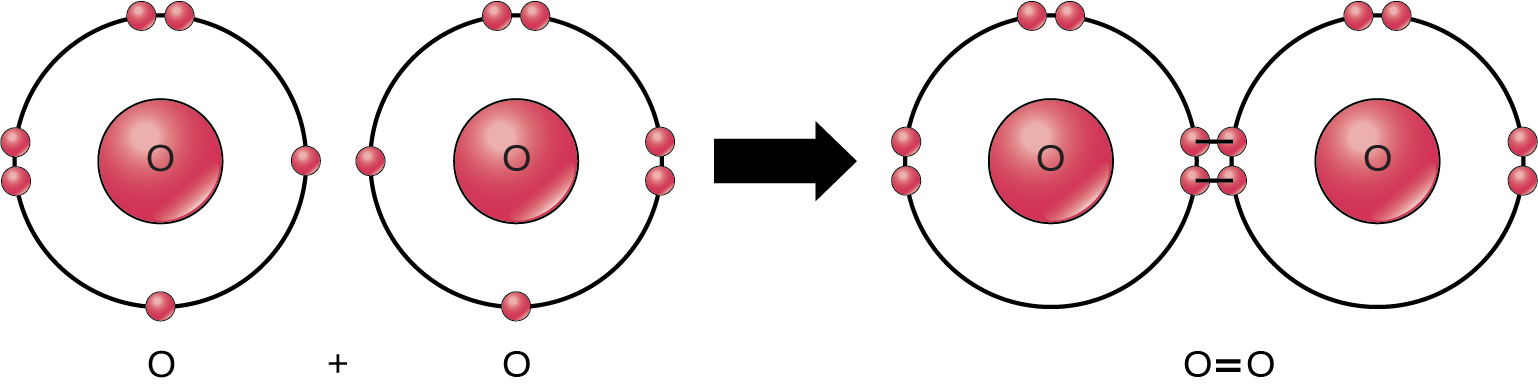
Some chemical reactions, such as the one above, can proceed in one direction until they expend all the reactants. The equations that describe these reactions contain a unidirectional arrow and are irreversible. Reversible reactions are those that can go in either direction. In reversible reactions, reactants turn into products, but when the product’s concentration goes beyond a certain threshold (characteristic of the particular reaction), some of these products convert back into reactants. At this point, product and reactant designations reverse. This back and forth continues until a certain relative balance between reactants and products occurs—a state called equilibrium. A chemical equation with a double headed arrow pointing towards both the reactants and products often denote these reversible reaction situations.
For example, in human blood, excess hydrogen ions (H+) bind to bicarbonate ions (HCO3–) forming an equilibrium state with carbonic acid (H2CO3). If we added carbonic acid to this system, some of it would convert to bicarbonate and hydrogen ions.
HCO3− + H+ ⇌ H2CO3
However, biological reactions rarely obtain equilibrium because the concentrations of the reactants or products or both are constantly changing, often with one reaction’s product a reactant for another. To return to the example of excess hydrogen ions in the blood, forming carbonic acid will be the reaction’s major direction. However, the carbonic acid can also leave the body as carbon dioxide gas (via exhalation) instead of converting back to bicarbonate ion, thus driving the reaction to the right by the law of mass action. These reactions are important for maintaining homeostasis in our blood.
HCO3− + H+ ⇌ H2CO3 ⇌ CO2 + H2O
Ionic Bonds
Some atoms are more stable when they gain or lose an electron (or possibly multiple electrons) and form ions. The gain or loss of electron(s) results in a filled valence shell and makes them energetically more stable. Because the number of electrons does not equal the number of protons, each ion has a net charge. Cations are positive ions that form by losing electrons. Negative ions form by gaining electrons, which we call anions. We designate anions by their elemental name and change the ending to “-ide”, thus the anion of chlorine is chloride, and the anion of sulfur is sulfide. A general rule is that, to achieve a filled valence shell, metals tend to lose electrons and nonmetals tend to gain electrons.
Scientists refer to this movement of electrons from one element to another as electron transfer. As Figure 2.10 illustrates, sodium (Na) only has one electron in its outer electron shell. It takes less energy for sodium to donate that one electron than it does to accept seven more electrons to fill the outer shell. If sodium loses an electron, it now has 11 protons, 11 neutrons, and only 10 electrons, leaving it with an overall charge of +1. We now refer to it as a sodium ion. Chlorine (Cl) in its lowest energy state (called the ground state) has seven electrons in its outer shell. Again, it is more energy-efficient for chlorine to gain one electron than to lose seven. Therefore, it gains an electron to create an ion with 17 protons, 17 neutrons, and 18 electrons, giving it a net negative (–1) charge. We now refer to it as a chloride ion. In Figure 2.10, sodium will donate its one electron to empty its shell, and chlorine will accept that electron to fill its shell. Both ions now satisfy the octet rule and have complete outermost shells. Note that these transactions can normally only take place simultaneously: in order for a sodium atom to lose an electron, it must be in the presence of a suitable recipient like a chlorine atom. After the electron transfer, the positively charged sodium ions and negatively charged chloride ions are held together by electrostatic attraction, ionic bonds, to make crystals of sodium chloride, or table salt, creating a crystalline solid with zero net charge. Substances that are made of ions are called ionic compounds.
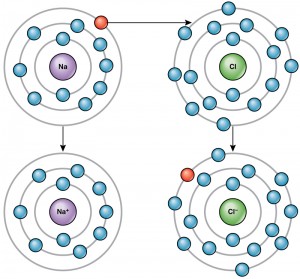
Physiologists refer to certain salts (ionic compounds) as electrolytes. Sodium, potassium, and calcium ions are necessary for nerve impulse conduction, muscle contractions, and water balance. Many sports drinks and dietary supplements provide these ions to replace those lost from the body via sweating during exercise.
Covalent Bonds
A type of strong chemical bond between two or more atoms is a covalent bond. These bonds form when a pair of electrons is shared between two elements and are the strongest and most common form of chemical bond in living organisms. Covalent bonds form between the elements that make up the biological molecules in our cells.
The hydrogen and oxygen atoms that combine to form water molecules are bound together by covalent bonds. Each covalent bond represents a pair of electrons shared between the two bonded atoms. The bonding pair of electrons resides partly in the valence shell of the hydrogen atom and partly in the valence shell of the oxygen atom. To fill the valence shell of the oxygen atom, two additional electrons are needed to achieve an octet, hence, two hydrogen atoms are needed to bond to oxygen. This sharing of electrons is a lower energy state for all of the bonding atoms involved than if the atoms existed without their valence shells filled.
The uneven sharing of electrons between atoms give rise to dipole moments in a molecule, where a region of the molecule will be slightly positive (δ+, lower electron density) and another region will be slightly negative (δ-, greater electron density) (Figure 2.11). In a polar covalent bond, the electrons shared by the atoms spend more time closer to one nucleus than to the other nucleus. Because of the unequal distribution of electrons between the different nuclei, a slightly positive (δ+) and slightly negative (δ–) charge develops. The covalent bonds between hydrogen and oxygen atoms in water are polar covalent bonds. The shared electrons spend more time near the oxygen nucleus, giving it a small negative charge, than they spend near the hydrogen nuclei, giving these atoms a small positive charge.
View this short video to see an animation of ionic and covalent bonding.
Nonpolar covalent bonds form between two atoms of the same element or between different elements with similar electronegativities. For example, molecular oxygen (O2) is nonpolar because the electrons distribute equally between the two oxygen atoms. Two covalent bonds form between the two oxygen atoms because oxygen requires two shared electrons to fill its outermost shell. Nitrogen molecule will form three covalent bonds (also called triple bond) between two atoms of nitrogen because each nitrogen atom needs three electrons to fill its outermost shell. Figure 2.11 shows another example of a nonpolar covalent bond—the C-H bond of methane (CH4). Carbon has four electrons in its outermost shell and needs four more for an octet. Carbon obtains these four electrons from four hydrogen atoms, each hydrogen atom sharing one electron with carbon to form the C-H bond. Similarly, the carbon atom also shares one electron to each C-H bond and completes the duet in the valence shell of each hydrogen atom. Carbon and hydrogen have similar electronegativities; thus, nonpolar bonds form. These elements share the electrons equally among the carbons and the hydrogen atoms, creating a nonpolar covalent molecule.
Despite the bonding of atoms of different electronegativities, the molecule may be overall nonpolar. In the case of the carbon dioxide molecule (Figure 2.11), the C-O bond is polar. The carbon atom at the centre of the molecule is slightly positive and the oxygen atoms at the ends are slightly negative. Due to the geometry of the molecule, the dipole moment in one C-O bond counteracts that of the other C-O bond and results in a net zero dipole moment, a nonpolar molecule.
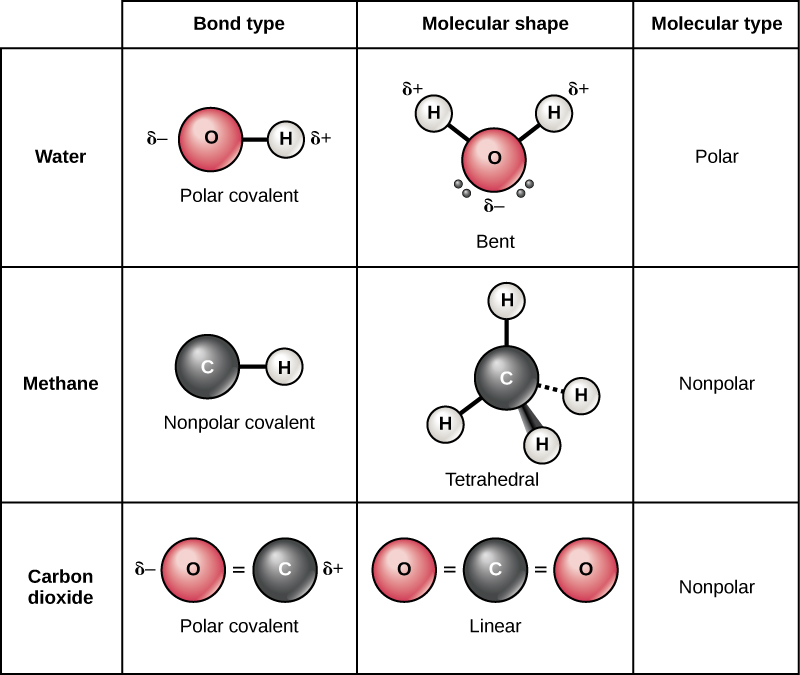
Hydrogen Bonds
Ionic and covalent bonds are strong bonds that require considerable energy to break. However, not all bonds between elements are ionic or covalent bonds. Weaker bonds can also form. These are intermolecular attractions that occur between partial positive and negative charges that do not require much energy to break. Two weak bonds that occur frequently are hydrogen bonds and van der Waals interactions. These bonds give rise to the unique properties of water and the unique structures of DNA and proteins.
When a hydrogen atom is bonded to an electronegative atom, such as nitrogen, oxygen, or fluorine, the hydrogen atom in that bond has a partial but substantial positive charge. This is because the shared electron is pulled more strongly toward the other element and away from the hydrogen nucleus. Because the hydrogen atom is slightly positive (δ+), it will be attracted to neighboring negative partial charges (δ–) on another nitrogen, oxygen, or fluorine atom. When this happens, a weak interaction occurs between the δ+ charge of the hydrogen atom of one molecule and the δ– charge of the other molecule. This interaction is called a hydrogen bond. This type of bond is common; for example, the liquid nature of water is caused by the hydrogen bonds between water molecules (Figure 2.12). Hydrogen bonds give water the unique properties that sustain life. If it were not for hydrogen bonding, water would be a gas rather than a liquid at room temperature.
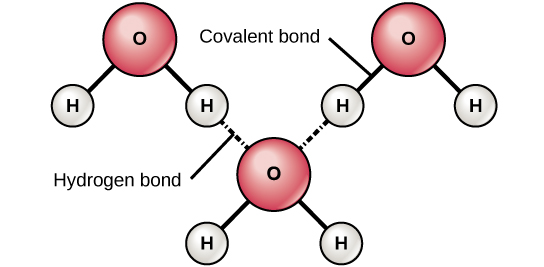
Hydrogen bonds can form between different molecules and they do not always have to include a water molecule. For example, hydrogen bonds hold together two long strands of DNA to give the DNA molecule its characteristic double-stranded structure. Hydrogen bonds are also responsible for some of the three-dimensional structure of proteins.
van der Waals Interactions
Like hydrogen bonds, van der Waals interactions are weak attractions or interactions between molecules. They occur between polar, covalently bound, atoms in different molecules. These weak attractions are caused by temporary partial charges formed when electrons move around a nucleus.
CAREER CONNECTION
Radiography Technicians
Have you or anyone you know ever had a magnetic resonance imaging (MRI) scan, a mammogram, or an X-ray? These tests produce images of your soft tissues and organs (as with an MRI or mammogram) or your bones (as happens in an X-ray) by using either radio waves or special isotopes (radiolabeled or fluorescently labeled) that are ingested or injected into the body. These tests provide data for disease diagnoses by creating images of your organs or skeletal system.
MRI imaging works by subjecting hydrogen nuclei, which are abundant in the water in soft tissues, to fluctuating magnetic fields, which cause them to emit their own magnetic field. This signal is then read by sensors in the machine and interpreted by a computer to form a detailed image.
Some radiography technologists and technicians specialize in computed tomography, MRI, and mammography. They produce films or images of the body that help medical professionals examine and diagnose. Radiologists work directly with patients, explaining machinery, preparing them for exams, and ensuring that their body or body parts are positioned correctly to produce the needed images. Physicians or radiologists then analyze the test results.
Radiography technicians can work in hospitals, doctors’ offices, or specialized imaging centres. Training to become a radiography technician happens at hospitals, colleges, and universities that offer certificates, associate’s degrees, or bachelor’s degrees in radiography.
Pharmaceutical Chemist
Pharmaceutical chemists are responsible for developing new drugs and trying to determine the mode of action of both old and new drugs. They are involved in every step of the drug development process. We can find drugs in the natural environment or we can synthesize them in the laboratory. In many cases, chemists change naturally occurring compounds in the laboratory to make them safer and more effective, and sometimes synthetic versions of drugs substitute for the version we find in nature.
After a drug’s initial discovery or synthesis, the chemist then develops the drug, perhaps chemically altering it, testing it to see if it is toxic, and then designing methods for efficient large-scale production. Then, the process of approving the drug for human use begins. In the United States, the Food and Drug Administration (FDA) handles drug approval. This involves a series of large-scale experiments using human subjects to ensure the drug is not harmful and effectively treats the condition for which it is intended. This process often takes several years and requires the participation of physicians and scientists, in addition to chemists, to complete testing and gain approval.
An example of a drug that was originally discovered in a living organism is paclitaxel (Taxol), an anti-cancer drug used to treat breast cancer. This drug was discovered in the bark of the Pacific yew tree. Another example is aspirin, originally isolated from willow tree bark. Finding drugs often means testing hundreds of samples of plants, fungi, and other forms of life to see if they contain any biologically active compounds. Sometimes, traditional medicine can give modern medicine clues as to where to find an active compound. For example, people have used willow bark to make medicine for thousands of years, dating back to ancient Egypt. However, it was not until the late 1800s that scientists and pharmaceutical companies purified and marketed the Aspirin molecule, acetylsalicylic acid, for human use.
Occasionally, drugs developed for one use have unforeseen effects that allow usage in other, unrelated ways. For example, scientists originally developed the drug minoxidil (Rogaine) to treat high blood pressure. When tested on humans, researchers noticed that individuals taking the drug would grow new hair. Eventually the pharmaceutical company marketed the drug to men and women with baldness to restore lost hair.
Finally, a pharmaceutical chemist may discover negative effects or even lack of effects. In the early 1960s, inventors, doctors, and even a U.S. senator claimed anti-cancer properties of a new drug, Krebiozen, and began to market and sell it aggressively. Through the process of infrared spectrometry, FDA chemist Alma Levant Hayden and her team discovered that the “miracle drug” was nothing more than a common compound called creatine. A pharmaceutical chemist’s career may involve detective work, experimentation, and drug development, all with the goal of making human beings healthier.
Section Summary
Exercises
Glossary
anion: a negative ion formed by gaining electrons
atom: the smallest unit of matter that retains all of the chemical properties of an element
atomic mass: calculated mean of the mass number for an element’s isotopes
atomic number: the total number of protons in an atom
balanced chemical equation: statement of a chemical reaction with the number of each type of atom equalized for both the products and reactants
cation: a positive ion formed by losing electrons
chemical bond: an interaction between two or more of the same or different elements that results in the formation of molecules
chemical reaction: the breaking and/or forming of chemical bonds
chemical reactivity: the ability of substances to combine and to chemically bond with each other
compound: substances composed of at least two different elements
covalent bond: a type of strong bond between two or more of the same or different elements; forms when electrons are shared between elements
electrolyte: ion necessary for nerve impulse conduction, muscle contractions, and water balance
electron: a negatively charged subatomic particle that resides outside of the nucleus in the electron orbital; lacks functional mass and has a charge of –1
electron configuration: arrangement of electrons in an atom’s electron shell, for example 1s22s22p6
electron orbital: how electrons are spatially distributed surrounding the nucleus; the area where we are most likely to find an electron
electron transfer: the movement of electrons from one element to another; important in forming ionic bonds
electronegativity: ability of an atom to attract electrons shared in a covalent bond
element: one of 118 unique substances that cannot be broken down into smaller substances and retain the characteristic of that substance; each element has a specified number of protons and unique properties
equilibrium: steady-state of relative reactant and product concentration in reversible chemical reactions in a closed system
hydrogen bond: a hydrogen atom that is bonded to nitrogen, oxygen, or fluorine will have a partial positive charge that is attracted to the partial negative charge on another atom of nitrogen, oxygen, or fluorine
inert gas (also, noble gas): element with filled outer electron shell that is unreactive with other atoms
ion: an atom or compound that does not contain equal numbers of protons and electrons, and therefore has a net charge
ionic bond: a chemical bond that forms between ions of opposite charges
irreversible chemical reaction: chemical reaction where reactants proceed unidirectionally to form products
isotope: one or more forms of an element that have different numbers of neutrons
law of mass action: chemical law stating that the rate of a reaction is proportional to the concentration of the reacting substances
mass number: the number of protons plus neutrons in an atom
matter: anything that has mass and occupies space
molecule: two or more atoms covalently bonded together
neutron: a subatomic particle with no charge that resides in the nucleus of an atom; has a mass of 1 amu
nonpolar covalent bond: a type of covalent bond that forms between atoms when electrons are shared equally between atoms, resulting in no regions with partial charges as in polar covalent bonds
nucleus: (chemistry) the dense center of an atom made up of protons and (except in the case of a hydrogen atom) neutrons
octet rule: states that the outermost shell of an element with a low atomic number can hold eight electrons
orbital: region surrounding the nucleus; contains electron(s)
periodic table of elements: an organizational chart of elements, indicating the atomic number and mass number of each element; also provides key information about the properties of elements
polar covalent bond: a type of covalent bond in which electrons are pulled toward one atom and away from another, resulting in slightly positive and slightly negative charged regions of the molecule
product: species resulting from a chemical reaction
proton: a positively charged subatomic particle that resides in the nucleus of an atom; has a mass of 1 amu and a charge of +1
radioactive isotope: an isotope that spontaneously emits particles or energy to form a more stable element
reactant: species participating in a chemical reaction
reversible chemical reaction: chemical reaction that functions bidirectionally, where products may turn into reactants if their concentration is great enough
valence shell: outermost shell of an atom or ion
van der Waals interaction: a weak attraction or interaction between molecules caused by slightly positively charged or slightly negatively charged atoms
Media Attribution
- Figure 2.7 by Bill Faulkner/NPS